Will artificial photosynthesis ever see the light of day?
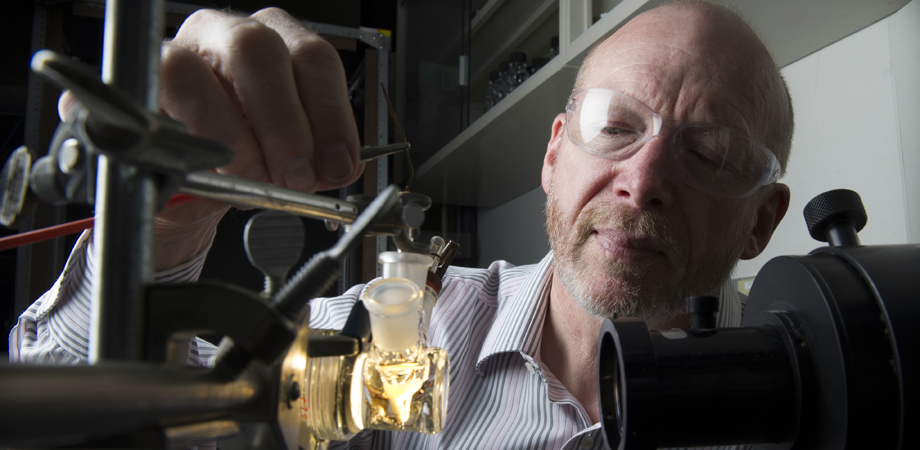
In 1912, Italian photochemist Giacomo Ciamician laid out a remarkable vision of a world powered by the Sun.
"On the arid lands there will spring up industrial colonies without smoke and without smokestacks," Ciamician wrote in a paper published in Science, "forests of glass tubes will extend over the plains and glass buildings will rise everywhere; inside of these will take place the photochemical processes that hitherto have been the guarded secret of the plants, but that will have been mastered by human industry which will know how to make them bear even more abundant fruit than nature, for nature is not in a hurry and mankind is."
Coal fueled the Industrial Revolution, but Ciamician had already spotted a problem: though stores were vast, they weren't inexhaustible. (He said nothing about the environmental consequences of mining and burning coal, an insight for a later time.) "Modern civilization is the daughter of coal," he wrote, "for this offers to mankind the solar energy in its most concentrated form ...."
What solar lacked in energy density it more than made up for in abundance, he argued. By his calculations, the Sahara Desert received a daily dose of solar energy equivalent to that contained in six billion tons of coal. All that was necessary was to harness it the way plants do.
More than a century later, Ciamician's future is both tantalizingly close and frustratingly elusive. Solar energy is a reality in ways he could not have predicted. But the Sun still has an inconvenient tendency to set every day. Plants don't wither and die at night because they've converted sunlight, water, and carbon dioxide into chemical bonds, glucose in this case, to fuel growth.
The ability to do the same thing, with the same ingredients, is the holy grail of renewable energy. Instead of glucose, artificial photosynthesis would produce hydrogen or a liquid hydrocarbon that could serve as a direct replacement for liquid fossil fuels, using infrastructure already in place. Electric vehicles are a part of the carbon-free energy future, but batteries won't work everywhere. Passenger planes, ships, and trains need energy-dense liquid fuel; likewise, you'll probably never heat your home with a battery-powered furnace.
We are indeed in a hurry to replace fossil fuels. Now, science just has to make nature's secrets bear fruit.
Success and Silence
Though discovery of the photovoltaic effect dates to 1839, and Bell Labs researchers built the first photovoltaic cell that generated enough electricity to run a device in 1954, study of artificial photosynthesis really began in 1972 when University of Tokyo researcher Akira Fujishima and Kenichi Honda successfully split water into hydrogen and oxygen using only sunlight as the source of energy.
Three years later, Fujishima and colleagues reported sustained production of hydrogen from an electrochemical photocell with a titanium dioxide
(TiO2) crystal anode to produce oxygen, and a platinum cathode to produce hydrogen. The device made 1.1 liters of hydrogen per day in the August sun as it falls on Japan, an efficiency rate they calculated at 0.4 percent. That's less than half what nature achieves, but a promising start.
A perovskite solar cell integrated with an electrolyzer, where the high voltage is applied to water electrolysis to make hydrogen, a storable solar fuel. The small bubbles are the hydrogen being produced. Credit: Dennis Schroeder/NREL
Fujishima didn't report on his device's lifespan, but it's safe to assume it was short. One result of splitting water is that the water itself becomes corrosive and degrades or destroys the photoelectrodes. By 1980, Fujishima realized that while TiO2 alone wasn't the answer for a water-splitting device, it made a great coating for glass, tiles, and other surfaces exposed to sunlight, in effect making them self-cleaning. That's easily commercialized research, unlike artificial photosynthesis.
It would be a familiar pattern; exciting breakthroughs followed by silence as the scientific challenges came into focus. In 1998, John Turner with the National Renewable Energy Laboratory (NREL) demonstrated a device that extracted hydrogen from water at an eye-popping efficiency rate of 12.4 percent. His discovery generated an avalanche of news stories, 300 by one tally. The catch? Turner's device used rare and expensive materials, including the noble metal platinum as a catalyst. Turner's device cost an estimated $10,000 per square centimeter and only lasted 20 hours.
In 2011, Daniel Nocera, then a chemistry professor at MIT, made headlines with a device built out of readily available materials—mostly silicon, cobalt, and nickel—that split water at about 2.5 percent efficiency. Nocera envisioned home-based systems that collected hydrogen to power fuel cells and produce electricity, an elegant solution that could easily be deployed all over the developing world. He even formed a startup to move the idea forward. But the company eventually shelved the idea because it was too expensive to deploy as a technology, instead using some of the ideas behind it to develop batteries.
Nature makes photosynthesis look easy, but the artificial version is devilishly complicated. At minimum it requires a light absorber, catalysts, an electrolyte, and a way to keep the resulting products—oxygen and hydrogen—separate so they don't recombine into water or worse, explode. The reaction that produces oxygen turns water acidic, while the reaction that produces hydrogen turns water basic, so photoanodes and catalysts must be stable and efficient in those conditions. Systems designers have to consider electrical resistance, chemical transport, and varying timescales in which processes occur. Pieces of the device must be mutually compatible. And the whole thing should be simple and inexpensive.
New Marching Orders
These are problems worth solving. Hydrogen can power fuel cells in electric vehicles or be burned in a modified combustion engine. It can be used as a nonfossil chemical feedstock to make ammonia, vital for food production. It can be combined with carbon dioxide to make liquid hydrocarbon fuel via the Fischer-Tropsch process. Someday, scientists hope it will be the first part of an integrated device that takes in sunlight, water, and carbon dioxide and produces usable hydrocarbon fuel.
While some of the most exciting advances have been made by small teams working in a lab, it's become obvious that real success will require a major initiative with a clearly defined goal and big money backing it. A handful of countries, including the United States, have put major resources into the effort.
Enter the Joint Center for Artificial Photosynthesis (JCAP), a US Department of Energy (DOE) Innovation Hub funded in 2010 as part of President Barack Obama's push toward renewable energy. JCAP started with $122 million in funding over five years and brought together more than 200 scientists from Caltech, DOE's Lawrence Berkeley National Laboratory (Berkeley Lab), University of California campuses, and SLAC National Accelerator Laboratory. It had a clear goal to develop an integrated solar-energy-to-chemical-fuel conversion system and move it from the benchtop to commercialization.
Caltech's 2015 stand-alone water-splitting prototype included two chambers separated by a semi-permeable membrane that allowed collection of both gas products. Credit: Lance Hayashida/Caltech
Nathan Lewis, the George L. Argyros professor of chemistry at Caltech and JCAP's founding director, was confident that success was at hand. "We are going to build prototypes of solar fuel generators as soon as we can," he told the Los Angeles Times in a 2011 interview. "You will be able to hold it in your hand. It will look like bubble wrap, or it might look like a membrane, similar to the material in a very good waterproof jacket."
Lewis, who is well known in the field for his predictions of an energy-producing device that can be rolled out like artificial turf, even offered a timeline. "We are almost positive that the first prototype will not work. The second prototype will probably not work. But sometime, maybe by the sixth time, it will work. In the first five years, we'll have working protenterotypes, but the first ones will be expensive. We'll declare partial victory, but we can always invent ways to make them faster and cheaper."
As if on cue, JCAP announced in 2015 that they'd met their goal. By coating light-absorbing semiconductors such as silicon or gallium arsenide with a nanometer-scale skin of TiO2—they created photoelectrodes that were durable, efficient, and cheap. A two-nanometer layer of nickel on top of the TiO2 proved to be an effective catalyst for the photoanode which was grown onto a photocathode that used a nickel-molybdenum catalyst. A special plastic membrane kept the resulting gases separate but allowed ions to flow between the two sides. The prototype was a one centimeter square, 10 percent efficient, and lasted 40-plus hours—a record at the time. Was our clean-energy future finally here?
That's a rhetorical question. Despite demonstrating real progress toward splitting water to produce hydrogen, the DOE cut JCAP's funding by 40 percent in its second five-year period, and also changed its mission. Instead of moving water-splitting out of the lab and into the world, the new mission goes many steps beyond, to the difficult fundamental science of creating liquid solar fuels.
The mission change ultimately impacted the research team more than the reduction in funding. "We had a goal and a vision, and inspiration from President Obama to go do this moon shot," said Lewis, about their first mission to split water. "And then you get close to the Moon they say, ‘No, we're going to slingshot you out to Mars...' You know, how you split water is not the same thing as how you selectively reduce CO2. Not even close. Well, it literally was like taking the spacecraft when it was in lunar orbit and ready to find the lander and then diverting it. All hands on deck, we're going to Mars tomorrow."
"We were very close on the water-splitting side," Lewis added. "I think it's disappointing."
Beyond the Moon
"Mars" is a new $100-million initiative from DOE announced in July 2020. The money will fund two new research centers: the Liquid Sunlight Alliance (LiSA), led by Caltech in association with Berkeley Lab; and the Center for Hybrid Approaches in Solar Energy to Liquid Fuels (CHASE), led by the University of North Carolina at Chapel Hill. LiSA's goals are to tailor molecules and materials to better understand transport and activity, make discoveries in light excitation and catalytic processes, and develop models for evaluating the durability of components and interfaces.
CHASE is tasked with researching photoelectrodes that combine semiconductors for light absorption with molecular catalysts. "Mechanistic investigations will provide unparalleled depth in the understanding of the light-driven chemistry at material-molecule-solution interfaces," the researchers wrote in a recent paper outlining the center's approach.
Pairing the light-absorbing properties of semiconductor materials with the selective fuel-producing reactivity of molecular catalysts, CHASE will advance a new paradigm of liquid solar fuels generation. Credit: CHASE
One challenge is developing catalysts that are both active in creating fuel and selective in the fuel they create. While the only products from splitting water are oxygen and hydrogen, reducing carbon dioxide can produce a range of products, some useful and some less so. The trick, CHASE believes, is to combine catalysts to create a multistep reaction sequence, or cascade, that spits out the desired fuel.
It's extremely challenging science and it's going to take time. "We're not trying to do this to put it in a device in our five-year plan," said Jillian Dempsey, associate professor of chemistry at the University of North Carolina, Chapel Hill, and CHASE deputy director. "I'm not promising you liquid solar fuels in 10 years. I'm trying to be optimistic that in 10 years we're going to be ready for translational science with robust systems, because we will have addressed all of these fundamental challenges associated with selectivity and durability."
In other words, we are closer than ever to solar fuels, but still a long way away. We now understand this relatively "simple" process is anything but. And we know it's a solution whose time has come.
Giacomo Ciamician got that part, too.
"If our black and nervous civilization, based on coal, shall be followed by a quieter civilization based on the utilization of solar energy, that will not be harmful to progress and to human happiness," Ciamician wrote in his 1912 paper. "The photochemistry of the future should not however be postponed to such distant times; I believe that industry will do well in using from this very day all the energies that nature puts at its disposal. So far, human civilization has made use almost exclusively of fossil solar energy. Would it not be advantageous to make better use of radiant energy?"
Bob Whitby is a science writer based in Fayetteville, Arkansas.
Enjoy this article? Get similar news in your inbox |
|